Over the last decade, the marine industry has come under increasing pressure to reduce its contributions to air emissions, with a growing focus on greenhouse gas (GHG) reduction. The International Maritime Organization (IMO) is the United Nations’ specialized agency responsible for safe, secure and efficient shipping and the prevention of pollution from ships, and as such has had the task of developing a strategy aligned with the UN’s overall policies on climate change. This overall strategy was released in April 2018 as IMO Resolution MEPC.304(72). It calls for:
- reductions of CO2 emissions per transport work, as an average across international shipping, by at least 40 per cent by 2030, pursuing efforts towards 70 per cent by 2050; and
- reductions of total GHG emissions from international shipping by at least 50 per cent by 2050;
In both cases, those percentages are compared with 2008 baseline values. These are immensely challenging objectives. To meet them, the marine industry cannot just do the same things better – it must get ready to do things differently.
Ships are long-life, high value assets. Most commercial ships designed for international voyages have design lives of 20-25 years. Some ship, such as ferries, Great Lakes vessels and other specialized types can have 40+ years of life expectancy. Many government vessels including coast guard and naval assets also reach or exceed these levels. Therefore, for designers, builders and many owners, 2050 is essentially now, and 2030 has effectively come and gone.
Some progress has been made towards achieving the IMO’s goals and targets, but much remains to be done. The balance of this article reviews the steps that will need to be taken and some of the unknowns that will need to be confronted.
Progress to Date
Previous IMO work in the area of GHG reduction included the introduction of the Energy Efficiency Design Index (EEDI) for new ships, and the requirement for existing ships to develop Ship Energy Efficiency Management Plans (SEEMP). These became mandatory in 2013. EEDI targets are applied to most but not all major commercial vessel types, and ratchet downwards, requiring large vessels to have values up to 30-50 per cent below the average 2000-2010 performance by 2025, depending on ship size and type. Essentially, EEDI limits installed power in relation to the weight of cargo that can be carried.
At the same time, other IMO measures for environmental protection have had impacts on energy efficiency. The introduction of Emission Control Areas (ECAs) for sulphur and nitrogen oxides (SOx and NOx) has led to fuel switching, the use of scrubbers, and the introduction of selective catalytic reduction systems (SCRs) for NOx control. The 2020 ban on heavy fuel oils (HFO) with more than 0.5 per cent sulphur content has accelerated these trends. Bolt-on devices which help achieve compliance reduce fuel efficiency. Fuel switching from HFO to diesel increases GHG emissions somewhat – though changing to alternative fuels such as natural gas gives substantial benefits (see more below).
The shipping industry has been able to cope with all these measures to date with limited impact on operations, though at time of writing the full impacts of the 2020 low sulphur limit are not yet clear. The first two stages of EEDI implementation, requiring up to 20 per cent GHG reduction have been achieved by ships scheduled for building this year. However, achieving further reductions is becoming progressively more difficult, and reaching the IMO 2050 goals does not appear possible without more radical changes to design and/or operational approaches.
How Ships Use Energy
It is worth revisiting some basic aspects of naval architecture and marine engineering to understand how energy is used in ships and the potential for further increases in energy efficiency. This provides context for the IMO 2050 objectives.
Resistance
There are three aspects to the overall problem of moving a ship through the water. First is the resistance to moving the hull. There are three main components to this: friction, wave-making, and form drag. For a unit of volume that a vessel can carry, friction is reduced by reducing the surface area in contact with the water, which taken to the extreme would result in an impractical circle or a slightly more practical square brick. Bricks unfortunately have very poor wave-making and form drag performance. Tankers and bulk carriers, optimized for transporting large volumes, are approximately rectangular bricks. They have shaped bows and stern forms to improve form drag and wave-making, and they move at relatively slow speeds.
At the other extreme, vessels intended to optimize wave-making resistance, such as container ships, have more slender forms with hull angles and supplementary features tailored to reducing the energy transmitted into their radiating wave fields. However, despite this, they still have much lower transportation efficiencies than tankers and bulkers, due to the dramatic fashion in which wave-making energy loss increases with speed. This can be seen in Figure 1, which shows the IMO EEDI reference baselines. Putting 100,000 tonnes of containers onto a 2010 bulker design would allow this to meet the IMO 2050 container ship target, at the cost of much longer delivery times. The bulker itself does not have this type of “simple” option available.
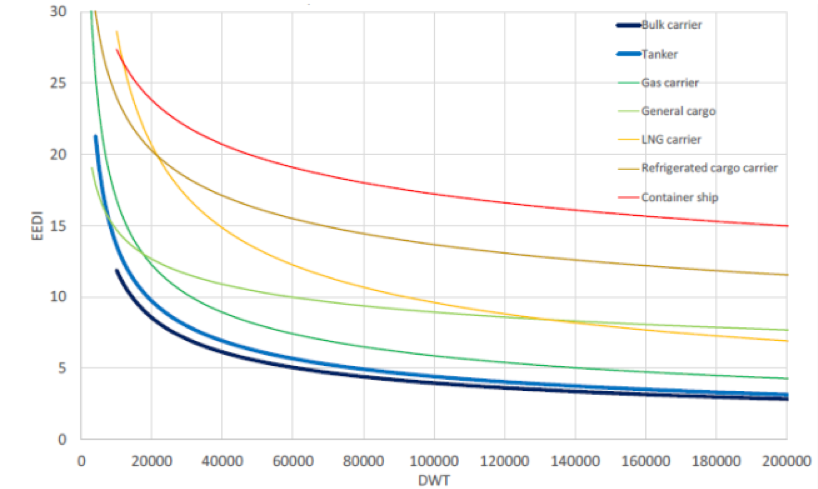
Figure 1: IMO EEDI Reference Lines (source IMO 2018)
Powering
Resistance is the minimum energy required to move the vessel. This energy must be supplied from some source. For millennia, vessels used wind as the main energy source, but over the last two centuries this has been supplanted, initially by steam and more recently by the ubiquitous diesel. These and other types of thermal engine use stored energy in some form to provide more reliable and higher power levels than those available from wind. The stored energy does not have to be a fossil fuel such as oil or coal, or indeed any form of hydrocarbon. However, the convenience of oil fuels means that these are the predominant transportation fuel source of the early 21st century.
Diesels are highly efficient thermal engines, with up to 55 per cent of the stored energy in the fuel being turned into useful work in the best marine diesels (well above the 40-45 per cent achieved by automotive diesels, and actually very comparable to current fuel cells). This is still somewhat below the theoretical maximum for the cycle of around 75 per cent, but is probably close to practical limits. Some of the energy lost to the engine exhaust and to cooling systems can be captured by waste heat recovery systems of various types, and these can add a few per cent to the overall energy efficiency of a ship. However, there are not huge gains to be made in peak efficiency. Larger improvements are possible in running diesels at lower loads, where efficiency has traditionally fallen off considerably. For most ship types, this represents a fairly small fraction of energy consumption.
Transmission
Power developed has to be applied in some way to provide motive force. In a sailing ship this transmission is directly through the rigging. For any vessel propelled by a thermal engine, the path is more complex. The simplest systems, and amongst the most efficient, involve slow-speed diesels driving a large propeller through a direct shaft. In this and most other ship applications, the largest energy losses are at the propulsor. Propeller efficiencies reach up to 70 per cent with good inflow and well-adapted designs, and it is difficult to improve on this for theoretical and practical reasons. Wake improvement devices can help improve poor forms, and contra-rotating propulsors can reduce energy losses in the water flow. Such devices are becoming increasingly popular.
Adding any transmission component between the engine and propulsor introduces energy losses. Mechanical transmissions with gearboxes and shafts can be 98+ per cent efficient. Electrical transmissions, with generators, converters, motors, etc., lose up to 10 per cent efficiency, and adding battery storage systems reduces this considerably through charging and discharging losses. Therefore, while modern hybrid systems can offer a great deal of operational flexibility for many ship types, they will not help with overall energy efficiency for most deep-sea vessels.
Decarbonization
Considering all the factors discussed above, there is limited potential to reduce the energy requirements for most shipping operations, other than by reducing speed. Reaching IMO 2050 goals will therefore require decarbonization of the energy supply, possibly in combination with a use of renewal hydrocarbons.
Methane, the main component of natural gas (NG), has the lowest carbon content of any hydrocarbon. This, plus the current low prices of natural gas, is a major factor in the decision of many ship operators to invest heavily in NG-fueled ships, generally with the fuel in liquid form (LNG) at cryogenic temperatures. Using LNG can reduce the EEDI by 15 per cent or more compared with conventional fuels, and is very effective in helping achieve compliance with IMO’s phase 1, 2 and 3 reduction targets. However, it cannot reach the 2050 targets and may be best considered as a transitional fuel, as is methanol.
Hydrogen is receiving increased attention as a potential transportation fuel. Some means of generating hydrogen (e.g. with renewable electricity sources) emit no greenhouse gases, and consuming hydrogen leads to few undesirable emissions. Like methane, hydrogen can be burnt in thermal engines using the Diesel or Otto cycles, and engines and systems developed for LNG are reasonably readily adaptable to hydrogen and to liquid or compressed hydrogen storage. Hydrogen is also a preferred fuel for fuel cells, in which chemical reactions, rather than combustion, generate energy. However, hydrogen is currently more expensive than hydrocarbon fuels, and cryogenic or compressed hydrogen systems are more expensive than those for LNG. Storage densities are very low, and fuel cell life expectancy is another drawback. Current projects are technology demonstrators rather than being commercially viable.
Other potential zero carbon fuels include ammonia, which does not need cryogenic or high pressure tanks, but which is toxic and corrosive. Used in thermal engines, it tends to create significant amounts of NOx, another harmful pollutant. It can also be used to drive fuel cells as a vehicle for the supply of hydrogen. A variety of hydrogen storage technologies are under development, but it is too soon to assess which of these may prove to be effective.
Of course, ships are traditional users of renewable energy, and wind and solar may be partial solutions to decarbonization. Their drawbacks are lack of reliability and energy density. The cloud of sails on a 19th century clipper ship would need to be far larger to provide powering for a large modern cruise or container ship; similarly, the available area for solar panels would need to be multiplied hugely to offer anything beyond an auxiliary power source. Integration of these technologies into designs that allow for efficient cargo handling and safe navigation is not simple, though variants such as the Flettner rotor (Figure 2) can represent more practical approaches for some ship types.
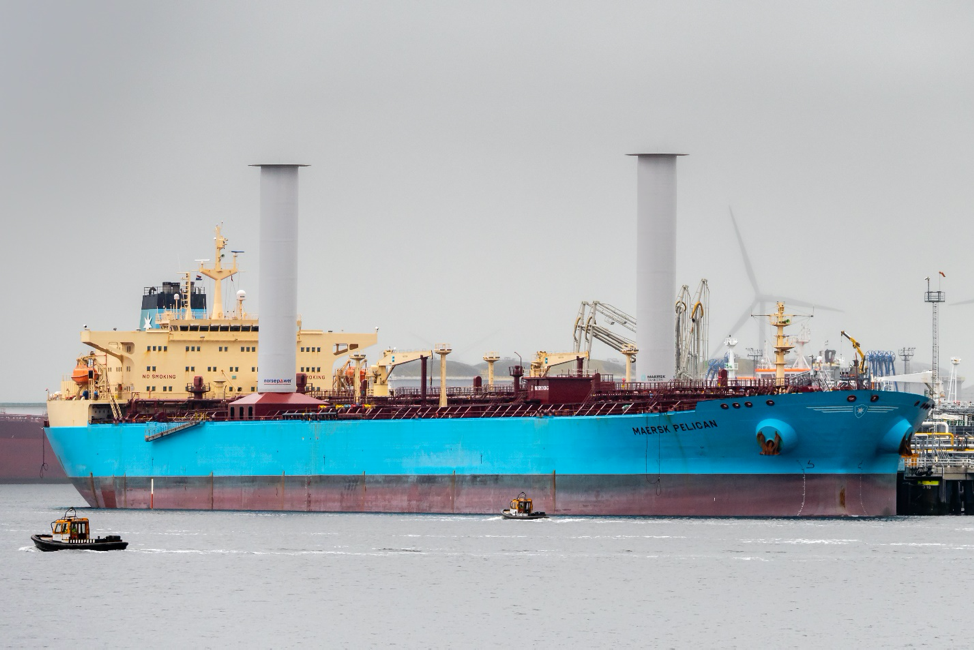
Figure 2: Norsepower Rotor on Maersk Tanker (courtesy Norsepower)
To summarize, there are no obvious complete solutions currently available that will allow commercial shipping to meet the IMO 2050 targets without fundamentally changing the nature of shipping operations. A group of leading industry organizations has proposed the set-up of a $5 billion research and development fund for work in this area, with a $2/tonne tax on all marine fuel to drive this. It is likely to be very difficult to get approvals for this at a global level, but something similar may be possible at a more regional level.
Canadian Issues
Canada’s economy is highly reliant on marine transportation and will certainly be impacted by the types of changes required by IMO 2050. There are also some challenges – and opportunities – where Canada’s position differs from most other countries.
The trend towards bigger ships with lower power will have significant impacts on the need for icebreaker services in Eastern and Arctic Canada, even as average ice cover continues to decline. The EEDI formulae include some adjustments for ice class, but designer/owner options for increasing efficiency are very limited, and often going more slowly in ice means stopping. Canadian Coast Guard’s future fleet requirements need to take this into consideration.
Meanwhile, Canada has several technology and resource advantages in a decarbonized or carbon neutral world. There are several strong fuel cell companies in Canada who are already in partnership with multi-national companies with marine interests. Renewable energy sources such as hydro-electricity and wind can be used to generate zero-emission hydrogen, and reforming hydrogen from methane – currently a significant source of gaseous hydrogen – can be “greened” through carbon capture. Biofuels are likely to retain at least a niche role, due to their advantages in energy density – for example, in military applications. This could be another area of competitive advantage for Canadians. Several premiers have been promoting modular nuclear reactors as a future power source, and these could potentially have many marine applications.
Whether Canada can use any of its advantages to become a leader in future marine technologies – or transportation technologies in general – will depend on whether we can succeed in creating a suitable ecosystem for innovation and development. This will be one of the overarching challenges between now and 2050.
Andrew Kendrick is the Vice President, Ottawa for Vard Marine Inc. He has a long and varied career as a naval architect, including ship design, applied research, and support to regulatory development at IMO and nationally. His recent projects include work on LNG, marine batteries, hydrogen and other future fuels. Andrew is also President-elect of the Society of Naval Architects and Marine Engineers (SNAME). Opinions expressed herein are personal and do not necessarily reflect those of Vard or SNAME.